
Tay Sachs Disease
Matt Regner
Introduction
Tay-Sachs disease (TSD) is a rare terminal genetic disorder characterized by the body’s inability to break down a certain lipid called GM2 ganglioside in brain cells. This is due to a mutation, or change in sequence, in the individual’s DNA within a gene called HEXA. The normal HEXA gene encodes a portion of a protein enzyme involved in ganglioside catabolism (degradation) and is responsible for breaking down GM2 ganglioside into GM3 ganglioside in neural cell lysosomes (1). Individuals with TSD that lack a functional copy of this enzyme called β-hexosaminidase A (β-HexA) are consequently unable to break down GM2 ganglioside (2). Thus, TSD is often referred to as a lysosomal storage disorder as GM2 ganglioside excessively accumulates in neural cell lysosomes.
Normally, gangliosides play a wide range of important physiological roles in the neural cell plasma membrane establishing cell-type-specific patterns on the outer surface, supporting cell-cell adhesions, and overall stabilization of axon-myelin interactions and the membrane itself (3,4,5). However, the excessive accumulation of GM2 ganglioside in TSD severely compromises normal lysosomal functioning. Lysosomes are vital cellular organelles that break down larger macromolecules into smaller units that the cell can reuse and recycle. In TSD, neural cell lysosomes become swollen membranous cytoplasmic bodies or lysosomal inclusions as they are unable to complete ganglioside catabolism (1). Unfortunately, the outcome is progressive neurodegeneration (neuron death), microglia-mediated inflammation and ultimately death in early life (6).
TSD was first described in the 1880s by two physicians, Warren Tay and Bernard Sachs, working independently. Tay identified the characteristic cherry red spot of the macula in the eyes of young TSD patients while Sachs characterized the familial patterns of TSD and found that cognitive development, reflex responses, and eyesight progressively decline until death in early childhood (7). Infantile TSD is the most common form of onset and the focus of this review. It often presents with delay in developmental milestones, low muscle tone and strength, impaired vision and hearing, and sometimes increased head size (8). Unfortunately, survival beyond the age four is uncommon (9). However, TSD may also present in juvenile or adult-onset forms with less severe symptoms due to residual β-hexosaminidase A activity and delayed neurodegeneration progression (10). A variety of therapeutic routes have been investigated for TSD yet there remains no cure.
Considering that only 10-15% of β-hexosaminidase A activity is required to prevent the buildup of GM2 ganglioside, a number of treatment strategies may seem promising (11). Some approaches involve reducing the amount of GM2 ganglioside (substrate-reduction therapy) to combat accumulation (12). Other strategies attempt to replace the defective enzyme via enzyme replacement therapy (13). Bone marrow transplantation and even gene therapy have also been investigated as therapeutic routes (14,15). However, each approach and their limitations will be further discussed below. Therefore, there should remain a strong research focus on TSD diagnostics and monitoring which will also soon be addressed below.
How Common is Tay Sachs Disease?
Although TSD exhibits a low prevalence in the general population, with a carrier frequency of 1 in 300, it is more common among individuals of Ashkenazi Jewish, Irish, French-Canadian and Cajun descents (16,17). If an individual is a carrier for a genetic disorder, it means they possess one mutant allele and one normal allele for the gene of interest. In the context of TSD, a carrier has one mutant copy and one normal copy of the HEXA gene. A carrier rate of 1 in 30 has been reported in Ashkenazi Jewish and French-Canadian populations while individuals of Irish of descent have shown a carrier frequency of 1 in 52 (18,19,20). An increased carrier rate for TSD, compared to the general population, was also reported in a Cajun population of Louisiana (21). Carrier individuals within these groups do not experience TSD as they possess one functional β-HexA enzyme encoded by one normal HEXA allele.
Tay Sachs Disease: a fatal defect in ganglioside metabolism
Gangliosides are highly abundant and important physiological players in the plasma membrane of neural cells (Fig. 1). Therefore, the nervous system is primarily affected by defects in ganglioside metabolism. In general, metabolism is comprised of anabolic (biosynthetic) and catabolic (degradative) sets of reactions. Biosynthesis of gangliosides is beyond the scope of this review, as Tay Sachs Disease (TSD) results from defects in ganglioside catabolism. Normal ganglioside catabolism begins with internalization from the membrane followed by travel through an endocytic pathway to arrive in the lysosome, the site of degradation (Fig. 2). Once incorporated into the lysosome, ganglioside degradation proceeds with a series of enzymes, including β-HexA, ultimately generating the functionally versatile lipid ceramide (Fig. 3A). TSD is characterized by the loss of β-HexA which results in excessive accumulation of its substrate GM2 ganglioside. In other words, the pathway does not proceed to completion with loss of β-HexA (Fig. 3B). The extent of GM2 ganglioside accumulation in lysosomes determines the pathological severity of TSD as lysosomes are vital organelles to the cell in terms of recycling materials (1). This loss of lysosomal function caused by GM2 ganglioside buildup has a detrimental impact in important regions of the nervous system each of which manifest the symptoms of TSD as discussed in the following section.
Symptoms of Tay Sachs Disease (8)


Fig. 1. A human neuron as a frame of reference.

Fig. 2. The endocytic pathway of ganglioside degradation. A variety of gangliosides decorate the outer surface of neural cells, but GT1a is used as an example for clarity. 1) GT1a is internalized from the membrane through a clathrin-independent manner (22, 23). 2) The endocytic vesicle carrying GT1a fuses with an early endosome. 3) Vesicle budding from early endosome fuses with a late endosome. 4) Vesicle budding from the late endosome fuses with the lysosome and incorporated into an intraendolysosomal luminal vesicle (ILV).

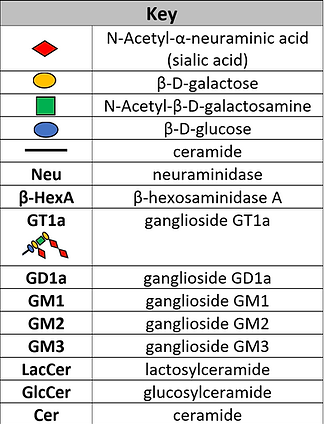
Fig. 3. Comparison of ganglioside metabolism between normal and TSD states. Enzyme names (black), activator proteins (blue), substrate names (red). (A) Ganglioside degradation mainly occurs at the intraendolysosomal luminal vesicle (ILV) membrane facing the lysosomal lumen (24). Neu1 removes the terminal sialic acid from GT1a to generate GD1a. Neu3/4 remove the terminal sialic acid from GD1a generating GM1. GM1 β-galactosidase with activator proteins GM2-AP and Sap-B remove the β-D-galactose from GM1 to generate GM2. β-hexosaminidase A (β-HexA) with activator GM2-AP removes N‐Acetyl-β-D-galactosamine from GM2 to generate GM3. Neu1/3/4 with activator Sap-B remove the last terminal sialic acid from GM3 to generate LacCer (lactosylceramide). β-galactosidase with activators Sap-B and Sap-C remove β-D-galactose from LacCer to generate GlcCer. β-glucocerebrosidase with activator Sap-C removes β-D-glucose from GlcCer (glucosylceramide) to generate ceramide (25). (B) Ganglioside degradation cannot proceed to completion in TSD. Neu1 removes the terminal sialic acid from GT1a to generate GD1a. Neu3/4 remove the terminal sialic acid from GD1a generating GM1. GM1 β-galactosidase with activator proteins GM2-AP and Sap-B remove the β-D-galactose from GM1 to generate GM2. β-HexA is non-functional in TSD. Therefore, the degradation pathway does not proceed and excessive accumulation of the substrate GM2 ganglioside occurs within the lysosome.
Linking GM2 ganglioside buildup to the symptoms of TSD
(Provided links in blue to encyclopedia references for relevant anatomical structures followed by reference)
Nervous system: GM2 ganglioside accumulates to such an extent that compromises lysosomal function. In other words, the buildup is so great that the lysosomes can no longer carry out their normal degradative activities. This severely impairs normal neural physiology leading to widespread neurodegeneration across different parts of the brain and spinal cord.
Loss of strength on one side of the body: GM2 ganglioside-mediated neurodegeneration in lateral corticospinal tract (26)
Delay/loss of developmental milestones: GM2 ganglioside-mediated neurodegeneration in cerebrum (27)
Uncoordinated and abnormal movement: GM2 ganglioside-mediated neurodegeneration in cerebellum (28)
Muscular: GM2 ganglioside accumulates to such an extent that compromises lysosomal function. Not surprisingly, an excess amount of lipids accumulate in the muscles as muscle cell lysosomes are unable to break them down. Neural physiology is also affected leading to neurodegeneration in neurons innervating muscle as well as the motor cortex.
Low muscle tone and strength: GM2 ganglioside-mediated neurodegeneration in motor cortex and innervating neurons (29)
Increased muscle lipid content: GM2 ganglioside-mediated impairment of muscle cell lysosomes

Fig. 4. Characteristic cherry red spot of the macula in TSD. GM2 ganglioside buildup in retinal ganglion cells, which are highly abundant in the macula but not the fovea, leads to enhanced contrast between macula and fovea. Adapted [reprinted] from “Beyond the cherry-red spot: Ocular manifestations of sphingolipid-mediated neurodegenerative and inflammatory disorders,” by Chen, H., Chan, A. Y., Stone, D. U., & Mandal, N. A., 2013, Survey of ophthalmology, 59(1), 64–76.
Eyes & Vision: Considering that eye tissue is an extension of neural tissue, vision is also affected by TSD. GM2 ganglioside accumulates to such an extent that compromises lysosomal function in cells of the optic nerve. This severely impairs normal neural physiology leading to neurodegeneration in the optic nerve which interferes with its ability to transmit visual sensory information. Additionally, GM2 ganglioside buildup in retinal ganglion cells lead to the appearance of a “cherry red spot” in the fundus or the back of the eye (Fig. 4).
Optic nerve deterioration: GM2 ganglioside-mediated neurodegeneration of the optic nerve
Cherry red spot of the macula: GM2 ganglioside accumulation in retinal ganglion cells surrounding the fovea (30)
Auditory: GM2 ganglioside accumulates to such an extent that compromises lysosomal function leading to neurodegeneration in auditory processing structures and regions.
Impaired hearing: GM2 ganglioside-mediated neurodegeneration of the auditory cortex and or auditory nerve (31)
Other: On a macroscopic scale, GM2 ganglioside accumulation contributes to macrocephaly/megalencephaly or enlargement of the head. GM2 ganglioside buildup not only mediates changes in cell volume, but also changes in brain tissue volume.
Abnormally large head: Accumulation of GM2 ganglioside leading to swelling of neural cells and enlargement of the brain (32)
Therapeutic and diagnostic approaches
Substrate reduction therapy (SRT)
SRT aims to reduce the rate of ganglioside biosynthesis with small molecules that can cross the blood brain barrier (12). Miglustat (N-butyldeoxynojirimycin, NB-DNJ) was first shown to reduce GM2 ganglioside accumulation in TSD rat models by 50% (33). NB-DNJ is a competitive inhibitor of glucosylceramide synthase which catalyzes the first step in glycosphingolipid synthesis (34). However, clinical trials involving five TSD patients determined that NB-DNJ was not effective in slowing the progression of neurodegeneration (35).
Enzyme replacement therapy (ERT)
ERT has demonstrated success as a treatment option for a number of lysosomal storage disorders (36, 37, 38, 39). However, ERT is not effective for disorders that affect the nervous system as the therapeutic enzymes are often unable to cross the blood brain barrier (40). Further complicating the development of an ERT treatment for TSD is the requirement of both β-HexA subunits (41). However, recent work engineered a hybrid μ subunit to form a μ-homodimer possessing the characteristics of both β-HexA subunits. This enzyme demonstrated favorable outcomes in vitro and in cellulo TSD models with intracranial administration (41).
Bone marrow transplantation (BMT)
BMT attempts to replace genetically diseased bone marrow stem cells. A clinical case utilizing BMT followed by SRT for TSD was reported with somewhat promising outcomes. Although further neurodegeneration was not prevented, a substantial increase in β-HexA activity was measured in leukocytes and plasma two years after transplantation (14).
Gene therapy
Challenging obstacles exist when it comes to TSD gene therapy, but promising results have been reported. Viral vectors have been the most common delivery method tested. A replication-defective herpes virus (HSV-1) was used to deliver a functional HEXA gene cDNA via intracranial injection in a TSD mouse model. The HSV-1 vector provided an extraordinary reduction in GM2 ganglioside accumulation throughout the mouse CNS demonstrating a high transduction efficiency (42). However, TSD gene therapy in humans utilizing this particular vector would most likely require several cDNA vector injections due to the increased size of the human brain (1). A number of other works have demonstrated success with adeno-associated virus (AAV)-based vectors, reporting improved mice survival rates (15,43,44,45). Yet AAV-based methods are limited by the small size of the virus and can only accommodate vector DNA up to 4.5 kb in length (41).
Diagnostics and disease monitoring
Future research should address diagnostic and monitoring strategies for TSD. It is important to be able to measure the extent of GM2 ganglioside accumulation for not only determining a prognosis but assessing response to therapy. Lyso-GM2 ganglioside, a close derivative of GM2 ganglioside that lacks a fatty acid tail, was reported as a potential biomarker measurable in human plasma samples. Lyso-GM2 ganglioside plasma levels of TSD patients were increased compared to normal control which correlated with decreased β-HexA activity (46). This could be used as a robust tool in the clinical setting as plasma samples can be easily collected. Quantitative MRI, as a non-invasive approach, was also reported to measure brain volume and volume changes in TSD patients demonstrating its potential use to assess disease progression (47). These innovative findings bring hope to those affected by TSD.
References
-
Solovyeva, V. V, Shaimardanova, A. A., Chulpanova, D. S., Kitaeva, K. V, Chakrabarti, L., & Rizvanov, A. A. (2018). New Approaches to Tay-Sachs Disease Therapy. Frontiers in Physiology. Retrieved from https://www.frontiersin.org/article/ 10.3389/fphys.2018.01663
-
Ferreira, C. R., & Gahl, W. A. (2017). Lysosomal storage diseases. Translational Science of Rare Diseases, 2(1–2), 1–71. https://doi.org/10.3233/TRD-160005
-
Kotani, M., & Tai, T. (1997). An immunohistochemical technique with a series of monoclonal antibodies to gangliosides: Their differential distribution in the rat cerebellum. Brain Research Protocols. https://doi.org/10.1016/S1385-299X(96)00025-6
-
Schnaar, R. L. (2016). Gangliosides of the Vertebrate Nervous System. Journal of Molecular Biology, 428(16), 3325–3336. https://doi.org/https://doi.org/10.1016/j.jmb.2016.05.020
-
Mori, K., Mahmood, M. I., Neya, S., Matsuzaki, K., & Hoshino, T. (2012). Formation of GM1 Ganglioside Clusters on the Lipid Membrane Containing Sphingomyeline and Cholesterol. The Journal of Physical Chemistry B, 116(17), 5111–5121. https://doi.org/10.1021/jp207881k
-
Wada, R., Tifft, C. J., & Proia, R. L. (2000). Microglial activation precedes acute neurodegeneration in Sandhoff disease and is suppressed by bone marrow transplantation. Proceedings of the National Academy of Sciences, 97(20), 10954 LP-10959. https://doi.org/10.1073/pnas.97.20.10954
-
Fernandes Filho, J. A., & Shapiro, B. E. (2004). Tay-Sachs Disease. Archives of Neurology, 61(9), 1466–1468. https://doi.org/10.1001/archneur.61.9.1466
-
Köhler, S., Gratian, D., Baynam, G., Palmer, R., Dawkins, H., Segal, M., Sullivan, K., et al. (2018). Expansion of the Human Phenotype Ontology (HPO) knowledge base and resources. Nucleic Acids Research, 47(D1), D1018–D1027. https://hpo.jax.org/app/browse/disease/ORPHA:845
-
Bley, A. E., Giannikopoulos, O. A., Hayden, D., Kubilus, K., Tifft, C. J., & Eichler, F. S. (2011). Natural history of infantile G(M2) gangliosidosis. Pediatrics, 128(5), e1233–e1241. https://doi.org/10.1542/peds.2011-0078
-
Kaback M.M., Desnick RJ. Hexosaminidase A Deficiency. (1999). In M.P. Adam, H.H. Ardinger, R.A. Pagon, et al. (Eds.), GeneReviews® [Internet]. Seattle (WA): University of Washington, Seattle; 1993-2019. https://www.ncbi.nlm.nih.gov/books/NBK1218/
-
Osher, E., Fattal-Valevski, A., Sagie, L., Urshanski, N., Sagiv, N., Peleg, L., Stern, N., et al. (2015). Effect of cyclic, low dose pyrimethamine treatment in patients with Late Onset Tay Sachs: an open label, extended pilot study. Orphanet Journal of Rare Diseases, 10, 45. https://doi.org/10.1186/s13023-015-0260-7
-
Platt, F.M., Jeyakumar, M., Anderson, U., Heare, T., Dwek, R.A., and Butters, T.D. (2003). Substrate reduction therapy in mouse models of the glycosphingolipidoses. Philosophical Transactions of the Royal Society of London. Series B: Biological Sciences, 358(1433), 947–954. https://doi.org/10.1098/rstb.2003.1279
-
Matsuoka, K., Tamura, T., Tsuji, D., Dohzono, Y., Kitakaze, K., Ohno, K., Itoh, K., et al. (2011). Therapeutic potential of intracerebroventricular replacement of modified human β-hexosaminidase B for GM2 gangliosidosis. Molecular Therapy : The Journal of the American Society of Gene Therapy, 19(6), 1017–1024. https://doi.org/10.1038/mt.2011.27
-
Jacobs, J. F. M., Willemsen, M. A. A. P., Groot-Loonen, J. J., Wevers, R. A., & Hoogerbrugge, P. M. (2005). Allogeneic BMT followed by substrate reduction therapy in a child with subacute Tay-Sachs disease. Bone Marrow Transplantation, 36, 925. Retrieved from https://doi.org/10.1038/sj.bmt.1705155
-
Gray-Edwards, H. L., Randle, A. N., Maitland, S. A., Benatti, H. R., Hubbard, S. M., Canning, P. F., Martin, D. R., et al. (2017). Adeno-Associated Virus Gene Therapy in a Sheep Model of Tay–Sachs Disease. Human Gene Therapy, 29(3), 312–326. https://doi.org/10.1089/hum.2017.163
-
Triggs-Raine, B. L., Mules, E. H., Kaback, M. M., Lim-Steele, J. S., Dowling, C. E., Akerman, B. R., et al. (1992). A pseudodeficiency allele common in non-Jewish Tay-Sachs carriers: implications for carrier screening. Am J Hum Genet.
-
Hoffman, J. D., Greger, V., Strovel, E. T., Blitzer, M. G., Umbarger, M. A., Kennedy, C., et al. (2013). Next-generation DNA sequencing of HEXA: a step in the right direction for carrier screening. Molecular Genetics & Genomic Medicine, 1(4), 260–268. https://doi.org/10.1002/mgg3.37
-
Petersen, G. M., Rotter, J. I., Cantor, R. M., Field, L. L., Greenwald, S., Lim, J. S., et al. (1983). The Tay-Sachs disease gene in North American Jewish populations: geographic variations and origin. American Journal of Human Genetics, 35(6), 1258–1269. Retrieved from https://www.ncbi.nlm.nih.gov/pubmed/6650504\
-
Palomaki, G. E., Williams, J., Haddow, J. E., & Natowicz, M. R. (1995). Tay-Sachs disease in persons of French-Canadian heritage in Northern New England. American Journal of Medical Genetics. https://doi.org/10.1002/ajmg.1320560412
-
Branda, K. J., Tomczak, J., & Natowicz, M. R. (2004). Heterozygosity for Tay-Sachs and Sandhoff Diseases in Non-Jewish Americans with Ancestry from Ireland, Great Britain, or Italy. Genetic Testing. https://doi.org/10.1089/1090657041797293
-
McDowell, G. A., Mules, E. H., Fabacher, P., Shapira, E., & Blitzer, M. G. (1992). The presence of two different infantile Tay-Sachs disease mutations in a Cajun population. American Journal of Human Genetics, 51(5), 1071–1077. Retrieved from https://www.ncbi.nlm.nih.gov/pubmed/1307230
-
Daniotti, J. L., & Iglesias-Bartolomé, R. (2011). Metabolic pathways and intracellular trafficking of gangliosides. IUBMB Life, 63(7), 513–520. https://doi.org/10.1002/iub.477
-
Crespo, P. M., Von Muhlinen, N., Iglesias-Bartolomé, R., & Daniotti, J. L. (2008). Complex gangliosides are apically sorted in polarized MDCK cells and internalized by clathrin-independent endocytosis. FEBS Journal. https://doi.org/10.1111/j.1742-4658.2008.06732.x
-
Möbius, W., Herzog, V., Sandhoff, K., & Schwarzmann, G. (1999). Intracellular Distribution of a Biotin-labeled Ganglioside, GM1, by Immunoelectron Microscopy After Endocytosis in Fibroblasts. Journal of Histochemistry & Cytochemistry, 47(8), 1005–1014. https://doi.org/10.1177/002215549904700804
-
Sandhoff, R., & Sandhoff, K. (2018). Emerging concepts of ganglioside metabolism. FEBS Letters, 592(23), 3835–3864. https://doi.org/10.1002/1873-3468.13114
-
Hawe, R. L., & Dewald, J. P. (2014). Assessment of the contralesional corticospinal tract in early-onset pediatric hemiplegia: Preliminary findings. Conference proceedings: Annual International Conference of the IEEE Engineering in Medicine and Biology Society. IEEE Engineering in Medicine and Biology Society. Annual Conference, 2014, 5336–5339. doi:10.1109/EMBC.2014.6944831
-
Mithyantha, R., Kneen, R., McCann, E., & Gladstone, M. (2017). Current evidence-based recommendations on investigating children with global developmental delay. Archives of Disease in Childhood, 102(11), 1071 LP-1076. https://doi.org/10.1136/archdischild-2016-311271
-
Schmahmann, J. D. (2004). Disorders of the Cerebellum: Ataxia, Dysmetria of Thought, and the Cerebellar Cognitive Affective Syndrome. The Journal of Neuropsychiatry and Clinical Neurosciences, 16(3), 367–378. https://doi.org/10.1176/jnp.16.3.367
-
O'Sullivan S. B. (2007). Strategies to Improve Motor Function. In S. B. O’Sullivan, & T. J. Schmitz (Eds.), Physical Rehabilitation (5th Ed.) Philadelphia: F.A. Davis Company.
-
Chen, H., Chan, A. Y., Stone, D. U., & Mandal, N. A. (2013). Beyond the cherry-red spot: Ocular manifestations of sphingolipid-mediated neurodegenerative and inflammatory disorders. Survey of ophthalmology, 59(1), 64–76. doi:10.1016/j.survophthal.2013.02.005
-
Behbehani R. (2007). Clinical approach to optic neuropathies. Clinical ophthalmology (Auckland, N.Z.), 1(3), 233–246.
-
Pavone, P., Praticò, A. D., Rizzo, R., Corsello, G., Ruggieri, M., Parano, E., & Falsaperla, R. (2017). A clinical review on megalencephaly: A large brain as a possible sign of cerebral impairment. Medicine, 96(26), e6814. doi:10.1097/MD.0000000000006814
-
Platt, F. M., Neises, G. R., Reinkensmeier, G., Townsend, M. J., Perry, V. H., Proia, R. L., et al. (1997). Prevention of lysosomal storage in Tay-Sachs mice treated with N- butyldeoxynojirimycin. Science. https://doi.org/10.1126/science.276.5311.428
-
Boomkamp, S. D., Rountree, J. S., Neville, D. C., Dwek, R. A., Fleet, G. W., & Butters, T. D. (2010). Lysosomal storage of oligosaccharide and glycosphingolipid in imino sugar treated cells. Glycoconjugate journal, 27(3), 297-308.
-
Maegawa, G. H., Banwell, B. L., Blaser, S., Sorge, G., Toplak, M., Ackerley, C., et al. (2009). Substrate reduction therapy in juvenile GM2 gangliosidosis. Molecular genetics and metabolism, 98(1-2), 215-224.
-
Connock, M., Burls, A., Frew, E., Fry-Smith, A., Juarez-Garcia, A., McCabe, C., ... & O'Hagan, A. (2006). The clinical effectiveness and cost-effectiveness of enzyme replacement therapy for Gaucher's disease: a systematic review.
-
Eng, C. M., Guffon, N., Wilcox, W. R., Germain, D. P., Lee, P., Waldek, S., ... & Desnick, R. J. (2001). Safety and efficacy of recombinant human α-galactosidase A replacement therapy in Fabry's disease. New England Journal of Medicine, 345(1), 9-16.
-
Klinge, L., Straub, V., Neudorf, U., & Voit, T. (2005). Enzyme replacement therapy in classical infantile pompe disease: results of a ten-month follow-up study. Neuropediatrics, 36(01), 6-11.
-
Wraith, J. E., Clarke, L. A., Beck, M., Kolodny, E. H., Pastores, G. M., Muenzer, J., ... & Braakman, T. (2004). Enzyme replacement therapy for mucopolysaccharidosis I: a randomized, double-blinded, placebo-controlled, multinational study of recombinant human α-L-iduronidase (laronidase). The Journal of pediatrics, 144(5), 581-588.
-
Sorrentino, N. C., D'orsi, L., Sambri, I., Nusco, E., Monaco, C., Spampanato, C., ... & Fraldi, A. (2013). A highly secreted sulphamidase engineered to cross the blood‐brain barrier corrects brain lesions of mice with mucopolysaccharidoses type IIIA. EMBO molecular medicine, 5(5), 675-690.
-
Tropak, M. B., Yonekawa, S., Karumuthil-Melethil, S., Thompson, P., Wakarchuk, W., Gray, S. J., ... & Mahuran, D. (2016). Construction of a hybrid β-hexosaminidase subunit capable of forming stable homodimers that hydrolyze GM2 ganglioside in vivo. Molecular Therapy-Methods & Clinical Development, 3, 15057.
-
Martino, S., Marconi, P., Tancini, B., Dolcetta, D., De Angelis, M. C., Montanucci, P., ... & Manservigi, R. (2005). A direct gene transfer strategy via brain internal capsule reverses the biochemical defect in Tay–Sachs disease. Human molecular genetics, 14(15), 2113-2123.
-
Cachón-González, M. B., Wang, S. Z., Ziegler, R., Cheng, S. H., & Cox, T. M. (2013). Reversibility of neuropathology in Tay–Sachs-related diseases. Human molecular genetics, 23(3), 730-748.
-
Osmon, K. J., Woodley, E., Thompson, P., Ong, K., Karumuthil-Melethil, S., Keimel, J. G., ... & Walia, J. S. (2016). Systemic gene transfer of a hexosaminidase variant using an scAAV9. 47 vector corrects GM2 gangliosidosis in Sandhoff mice. Human gene therapy, 27(7), 497-508.
-
Karumuthil-Melethil, S., Nagabhushan Kalburgi, S., Thompson, P., Tropak, M., Kaytor, M. D., Keimel, J. G., et al. (2016). Novel Vector Design and Hexosaminidase Variant Enabling Self-Complementary Adeno-Associated Virus for the Treatment of Tay-Sachs Disease. Human Gene Therapy, 27(7), 509–521. Retrieved from https://doi.org/10.1089/hum.2016.013
-
Kodama, T., Togawa, T., Tsukimura, T., Kawashima, I., Matsuoka, K., Kitakaze, K., … Sakuraba, H. (2011). Lyso-GM2 ganglioside: a possible biomarker of Tay-Sachs disease and Sandhoff disease. PloS one, 6(12), e29074. doi:10.1371/journal.pone.0029074
-
Nestrasil, I., Ahmed, A., Utz, J. M., Rudser, K., Whitley, C. B., & Jarnes-Utz, J. R. (2018). Distinct progression patterns of brain disease in infantile and juvenile gangliosidoses: Volumetric quantitative MRI study. Molecular Genetics and Metabolism, 123(2), 97–104. Retrieved from https://doi.org/10.1016 /j.ymgme.2017.12.432